The Genetic Self: Biological Age
Mitochondria are immortal, which is why they are critical to your long life
Biological vs. chronological age
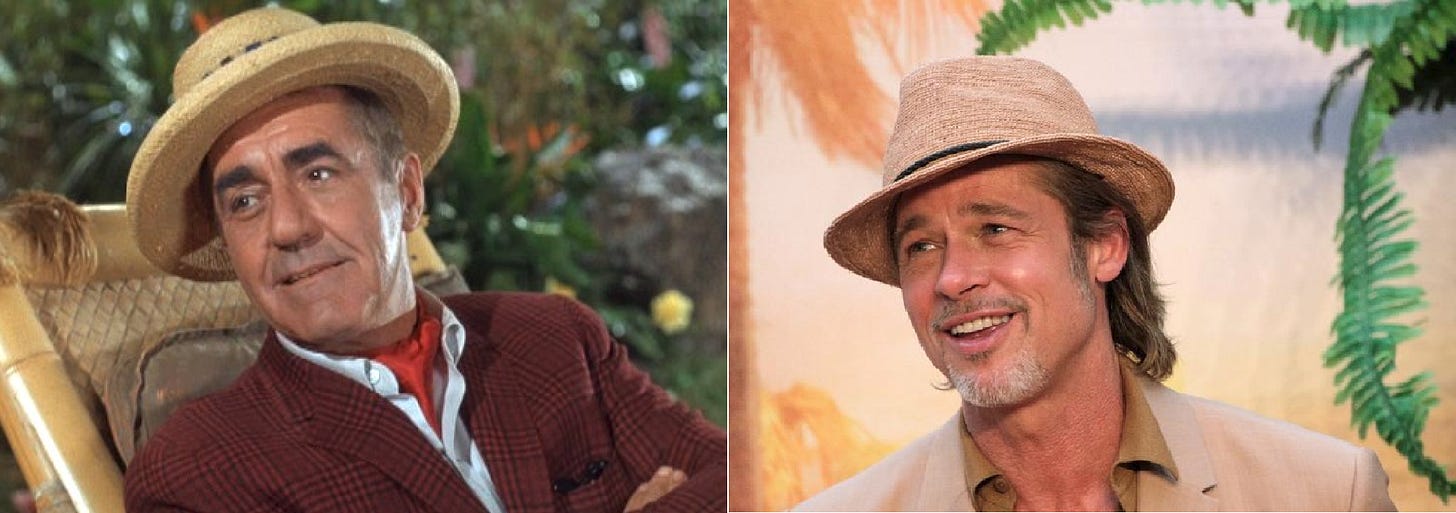
When I was a child in the 1970’s, the stereotypical television figure of a middle-aged white man was fat, grumpy, and old. Men like Ed Asner (Lou Grant on Mary Tyler Moore, age 41), Carol O’Connor (Archie Bunker, All in the Family, age 47), and Tom Bosley (Howard Cunningham, Happy Days, age 47) were all under 50 when they debuted in their signature roles, but in my childhood I thought of them all as ancient.
My own father was no better role model. Instead, he and my Mother complained about weight gain, creaking joints, and the occasional cognitive lapses they called “senior moments,” as if these experiences were an inevitable, unavoidable consequence of the growing number of days that had elapsed since they were born.
They’ve both passed on now.
Dad was morbidly obese and suffering the ill effects of several tumors, but he died peacefully in his sleep on his own terms at the age of 91. Mom’s circumstances were different. She lost her brain to dementia long before she died at the age of only 85. I buried her ashes last week, next to Dad’s, in the garden outside her church in Massachusetts.
I’m now 56 years old, and witnessing my parents’ decline has got me thinking about ageing, and how much different it is in some people than in others.
For example, Wilford Brimley started his Hollywood career in the 1960’s as a stuntman, but it really took off when he started playing old guys in the mid-1980’s. He turned 50 while filming Cocoon in 1985. The movie depicts what happens to three geriatric friends who seek to reverse ageing by stealing alien life force from their neighbor’s swimming pool, and Brimley was admittedly the youngest of the trio.
But did anyone recognize Brimley was only 49 the year before, when he portrayed Pop Fisher in The Natural?
Robert Redford is two years younger than Brimley:
The contrast we see on screen between these two actors illustrates the difference between biological and chronological age. Although LordOnlyKnows what they’re using besides make-up and wardrobe to appear an age that fits their roles, the point survives the dramatization.
In theory, if we knew our biological age, then we could use that information to tune our diet, exercise, and lifestyle to lead a longer, healthier, more vigorous and youthful life. That is, an accurate determination of biological age would be sensitive to behaviors or therapies that slow ageing and extend healthspan. Knowing your biological age could then be important for managing your health and your expectations of your future.
However, biological age is difficult to determine. There are over 300 theories of ageing, and most of them are unhelpful.
In truth, ageing is a complex phenomena that science has yet to fully decode. For example, ageing is not uniform among different animals, among different individuals, or even among different tissues in the body.
“Any modern theory of ageing should explain not only why organisms grow old with time and eventually die, but also, primarily, the evolutionary variations of life span and the reason why processes of ageing have such different rates in different species, different tissues, different organs, and different cells.”
— Medvedev 1990.
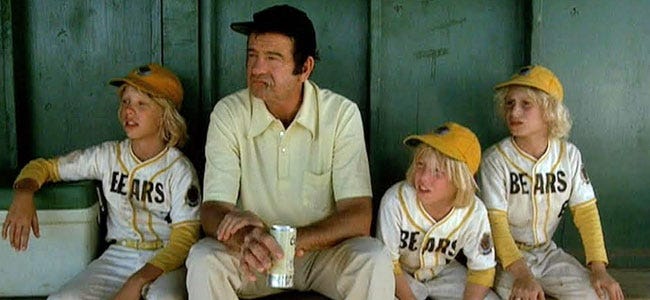
What is ageing?
Theories of ageing can generally be classified into three broad categories:
somatic theories that measure declining physiological parameters, like hand grip strength, walking speed, or time balancing on one leg.
genetic theories that measure biomarkers of accumulated damage to DNA, RNA, and/or the proteins they synthesize or the telomeres that protect them.
energetic or metabolic theories, including mitochondrial theories, that describe progressive declines in metabolic function.
None of these explain why we age. Rather, each theory is an attempt to improve upon the chronological measure of age so we can gain a better understanding of the mechanisms of ageing. If these theories worked, then we could endeavor to slow our biological clock by manipulating the variables that influence it.
But the first two of these theories do not work, and the third is difficult to measure.
The problem with somatic theories is that they confuse causation and correlation. We do not age because we lose hand grip strength. We lose hand grip strength because we age. While it might be a fine thing to improve our grip strength, it won’t lead us to the proverbial Fountain of Youth. The fact is that weight training both leads to stronger grip strength and better overall health. So it’s not that grip strength slows ageing. It’s that both grip strength and health improve with weight-bearing exercise.
Biochemical makers of accumulated DNA damage don’t work any better. For example, telomeres are protective nucleotides found at the end of chromosomes. As our cells divide and replicate, they gradually lose protective telomeres, so it stands to reason that longer telomeres will be found in younger people, and that the rate of telomere shortening would be related to ageing.
Except it’s not.
The correlation between telomere length and chronological age is poor, as is the correlation between biochemical markers of RNA function (transcriptomics), protein synthesis (proteomics), and metabolism. The only one of these five that demonstrates a good correlation to chronological age is DNA methylation (epigenetics), which I’ve written about before in ‘The Genetic Self: Epigenetics’.

The problem with epigenetics is that it does nothing to improve on chronological age as a predictor of expected healthspan, which defeats the whole purpose of estimating biological age in the first place.
There are two criteria that any effective measure of biological age must demonstrate:
For large statistical ensembles, it must correlate with chronological age.
For individuals, the departures from chronological age correlation must be predictive of expected healthspan. Put another way, wherever biological age departs from chronological age, the biological must be a better predictor of mortality than chronological.
Because the five biochemical markers reviewed in Jansen et al. (2021) fail one or both of these criteria, they’re ineffective measures of biological age.
I had my epigentic age measured anyway.
According to the Elysium Health ‘Index’ test, my biological age is 51 — five years younger than my chronological age. That feels good in the sense that it’s better than finding out that my DNA methylation is typical of someone older than me, but it doesn’t help me understand what I might do to take care better of myself.
Mitochondrial theories of age
Having ruled out the somatic and genetic theories of ageing, we are free to focus our attention on the energetic theories, which are much more promising.
Any energetic theory of the body must have its origins in mitochondria. In ‘The Genetic Self: Mitochondrial DNA’ I explained that mitochondria have their own DNA, independent from the DNA in your nucleus. Keeping this genetic information stored inside their own organelle walls allows the mitochondria to synthesize enzymes or other proteins right at the site where they are needed.
Because mitochondria are the site of energy conversion in the body, speed is essential when the body has to react fast.
All cells, with few exceptions (e.g., red blood cells) contain mitochondria to convert food energy like glucose and fats into electrical energy to power the body. For example, muscles cells have hundreds of mitochondria, because they demand so much energy. However, brown fat cells are packed with even more. Brown fat cells contain thousands of mitochondria to fuel cold thermogenesis — i.e., heat production to maintain body temperature during cold exposure.
Mitochondria are immortal
According to evolutionary theory, mitochondria were once independent prokaryotic organisms. Prokaryotes don’t have a nucleus inside their cells to protect their DNA (like eukaryotes have). At some point, some poor mitochondria became subsumed by a more complex, predatory eukaryotic cell. But instead of becoming digested, the prokaryotic mitochondria was incorporated as an organelle inside the cell membrane of the eukaryote. There it stayed, in mutualistic symbiosis, producing energy in the form of ATP from the food supplied by the increasingly complex and evolving multi-cellular eukaryotic organism.
While it’s easy to see why the eukaryote would want the more efficient energy mitochondria generating power for it, you might ask, “What’s in it for the mitochondria?”
But it’s important to keep in mind that prokaryotes, without a nucleus, do not reproduce like eukaryotes do.
In a sense, prokaryotes are immortal. They reproduce asexually by replicating their own DNA and dividing into two, identical cells that subsequently operate as separate organisms. In the absence of mutation, the DNA in a prokaryote can keep replicating itself for perpetuity.
By contrast, in complex, multi-cellular eukaryotes (e.g., human beings), cell division (mitosis) is a mechanism of growth, not reproduction. For example, sexual reproduction requires combining complementary strands of DNA from two parents, a Mother and a Father, to form a fertilized egg (zygote). Therefore, the offspring will always have a different genetic endowment than either parent. They are therefore recognizable as unique individuals distinct from their parents.
Only after successful sexual reproduction can mitosis proceed to grow a new organism into a new person with a unique genetic endowment. After sexual reproduction in a eukaryote, every cell in the new individual will have the same DNA, both nucleic and mitochondrial.
The problem of prokaryotic reproduction inside a eukaryotic human being is an evolutionary conundrum. Given that both the Mother’s eggs and the Father’s sperm carry different mitochondria, it’s not obvious how the zygote should carry out mitosis with two sets of donor mitochondria.
Evolution resolves this conundrum by destroying the Father’s mitochondria before the zygote begins cell division in earnest. Thus, we inherit our mitochondrial DNA exclusively from our Mother.
That means, absent mutation, our inherited mitochondria is identical to our Mother’s, and our Grandmother’s, and our ancient Great-great-great-grandmother’s, going all the way back to Eve — because prokaryotes are immortal in this way.
In practice, mutations are happening all the time. There are even cases of paternal mitochondria getting mixed up with maternal, confusing mitichondrial lineage (Brondham et al. 2003). Most of these aberrations are fatal, or result in disorders that make it very unlikely for the resulting human being to mature to reproductive success.
But not all of them.
So mitochondrial DNA are subject to their own selection pressures and do evolve over time — just not in the same way that the eukaryotic DNA inside our nucleus does.
Mitochondrial immortality would seem to rule them out as critical to ageing and lifespan in complex, multicellular eukaryotic creatures like humans. If the problem does not reside in the mitochondria, then it must be in other aspects of the eukaryotic organism, right?
Wrong.
How mitochondria hold the key to human longevity
Calorie restriction is the only reliable strategy for extending lifespan in laboratory-controlled animal models. Nothing else works as well, although some of the most promising contenders (e.g., rapamycin, Blagosklony 2019) also work energetically — i.e., on the metabolism.
What’s not yet clear is whether intermittent fasting and/or carbohydrate restriction can have the same beneficial, life-extending effect as calorie restriction.
It might, because carbohydrates are more dangerous to mitochondria than fats and proteins.
When we eat too many carbohydrates, the glucose levels in our bloodstream spike up. That glucose spike causes several things to happen, including a release of insulin from the islet cells of the pancreas.
Glucose cannot leave the bloodstream and enter cells without the benefit of insulin. So a glucose spike creates an insulin spike to help shuttle the glucose across the cell wall to get it to the mitochondria where it can be processed for either muscle energy (exercise), cold thermogenesis (heat production), or synthesis of lipids for storage in white fat cells.
As the mitochondria process the excess glucose, they inevitably create electron imbalances as a consequence of the additional energy conversion load. Those imbalances produce what are called reactive oxygen species (ROS, Turrens 2003). Under ordinary circumstances, the mitochondria produces metalloenzymes that will correct these electron imbalances by donating an electron to the ROS, reducing them to a more stable state.
Unfortunately for the mitochondria, when production of ROS exceeds the capacity of surrounding molecules to donate electrons, the ROS will find their way to mitochondrial DNA and cause damage. Without a nucleic membrane for protection, mitochondrial DNA are ten times more vulnerable to damage than nucleic DNA.
However, mitochondria protect themselves against DNA damage in several ways. First, they carry multiple copies of DNA so that they can select the best for use and reproduction. Second, they have mechanisms to repair damaged DNA, and other mechanisms to destroy mitochondria that are beyond repair.
Also, the body protects mitochondria from the damaging effects of glucose overload. For example, exercise fatigue is a way of resting the mitochondria without overheating them. And insulin resistance is a way of preventing excess glucose from reaching the mitochondria by keeping the glucose in the bloodstream until the mitochondria inside the cells are ready to process it.
Nonetheless, chronic carbohydrate overload will result in chronic mitochondrial damage. Ultimately, when the eukaryotic human being can no longer count on healthy mitochondria to provide energy conversion to support life, it dies.
All of which is to say that mitochondria may be immortal, but they are not invulnerable. They can be killed by overwork.
Life extension as death prevention?
Most of the leading causes of death in the United States result from metabolic disorders, including heart disease, most cancers, Alzheimer’s, obesity, high blood pressure (hypertension), and Type 2 diabetes.
There are several clinical names for what amounts to a problem of chronic carbohydrate overload. Sometimes it’s called metabolic syndrome, and sometimes hyperinsulinemia, or insulin resistance, or pre-diabetes.

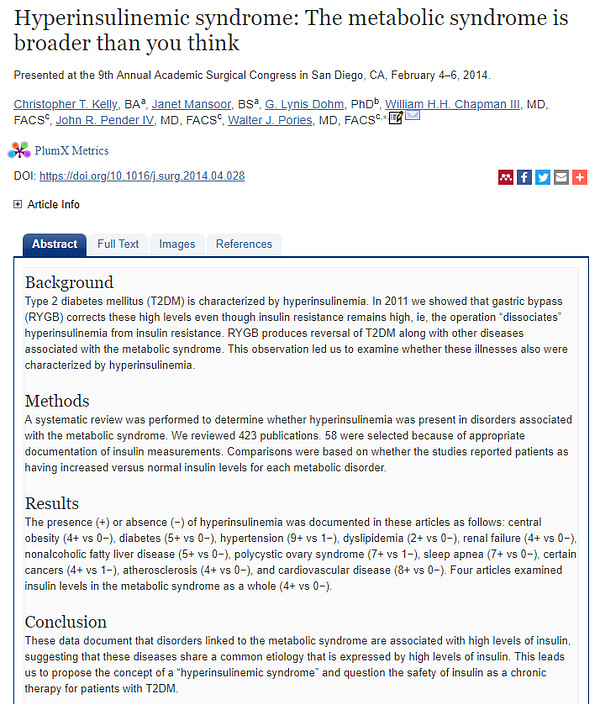
No matter what it’s called, the underlying condition is mitochondrial distress. The consequence is decreased health- and lifespan — in other words, reduced biological age.
In most cases, the mitochondria can be rescued by some combination of carbohydrate restriction, fasting, increased exercise, and deliberate cold exposure. Each of these alters the carbohydrate intake versus consumption balance in ways that give mitochondria time to repair and restore. For example, one study of German men diagnosed with Type 2 diabetes found that just ten days of mild cold exposure was sufficient to reduce insulin resistance by more than 40% (Hanssen et al. 2015), without changes in diet or exercise.
In those ten days, the German men who restored their mitochondrial function were biologically ageing in reverse.
Measuring your mitochondria
The field of mitochondrial-omics is less well developed than genomics, proteomics, transcriptomics (RNA), or metabolomics (Picard et al. 2019). Although the human mitochodrial genome has been sequenced, the fact that mitochondria keep multiple copies of their own DNA within their organelle walls can complicate analysis. The condition in which these multiple copies do not match is called heteroplasmy and it is especially evident in cancer cells, given that “primary dysregulation of mitochondrial function via mtDNA mutation is a pervasive feature of cancer” (Gammage & Frezza 2019). Nevertheless, “experimental approaches to determining a role for mtDNA mutations in cancer-associated mitochondrial dysfunction have yet to yield conclusive data, mostly because of the genetic intractability of the mitochondrial genome and consequently limited experimental tools.”
Mitochondrial sequencing is now available from commercial labs, although I haven’t yet tried them. In general, this approach is similar to other searches for biochemical markers like DNA methylation (epigenetics) and telomere length — except that it’s looking at mitochondrial markers, instead of nucleic.
Nonetheless, my sense is that looking for material markers may be the wrong approach. Given the role of mitochondria in energy conversion, it is likely that healthy mitochondria have an energetic signature different from unhealthy.
In a subsequent article, I’ll describe one estimate of my biological age based on the electrical field generated by my body that suggests my biological age is 30 years old.
And I’ll explain the reasons why that might not be too far off.